| |
from http://www.daviddarling.info/encyclopedia/E/Einstein_and_photoelectric_effect.html
Einstein and the photoelectric effect
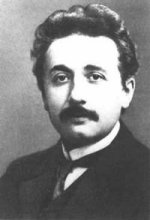 |
Albert Einstein
(1879-1955)
|
Mention Albert Einstein and the first thing that springs to mind is the
theory of relativity, that other extraordinary supernova that burst
upon 20th-century physics. Yet, incredibly, Einstein never won a Nobel
Prize for relativity. His one Nobel medal (he surely should have got at
least two), awarded in 1921 and presented in 1922, was for his
pioneering work in quantum theory. If Planck hadn't fathered quantum
theory (see
Max Planck and the origins of quantum theory) that role may well
have fallen to Einstein. As it was, Einstein was the first person to
take the physical implications of Planck's work seriously. The turning
point came when he saw how Planck's idea of energy quanta could be used
to account for some puzzling facts that had emerged about a phenomenon
known as the photoelectric effect.
Early studies of the photoelectric effect
In 1887, Heinrich Hertz became the first person to observe the
photoelectric effect during his experiments that confirmed Maxwell's
theory of electromagnetism. Hertz found that by shining
ultraviolet light onto metal electrodes, he could lower the voltage
needed to make sparks hop between the electrodes. The light obviously
had some electrical effect, but Hertz stopped short of speculating what
that might be. "I confine myself at present," he said, "to communicating
the results obtained, without attempting any theory respecting the
manner in which the observed phenomena are brought about."
In 1899 the English physicist J. J. Thomson offered an important clue
toward understanding the photoelectric effect. Thomson showed that
ultraviolet light, falling onto a metal surface, triggered the emission
of
electrons. These were tiny charged particles whose existence Thomson
had demonstrated a couple of years earlier and which he believed were
the only material components of atoms. The photoelectric effect, it
seemed to physicists at the time, must come about because electrons
inside the atoms in a metal's surface were shaken and made to vibrate by
the oscillating electric field of light waves falling on the metal. Some
of the electrons would be shaken so hard, the theory went, that
eventually they'd be tossed out altogether.
In 1902, Philipp Lenard, who'd earlier been an assistant to Hertz at the
University of Bonn, made the first quantitative measurements of the
photoelectric effect. He used a bright carbon arc light to study how the
energy of the emitted photoelectrons varied with the intensity of the
light and, by separating out individual colors, with the frequency of
light. Increasing the frequency of light, by selecting light from the
bluer end of the spectrum, caused the ejected electrons on average to be
more energetic, as predicted – because it was assumed they'd been made
to vibrate faster. Increasing the intensity of light (by moving the
carbon arc closer to the metal surface) caused more electrons to be
thrown out, also as expected. On the other hand, increasing the
intensity had no effect at all on the average amount of energy that each
ejected electron carried away. That came as a real shock. If, as
physicists believed, the photoelectric effect followed from an
interaction between electrons and electromagnetic waves, then
intensifying the radiation ought to shake the electrons in the metal
surface harder and so shoot them out with more energy. It was a mystery
why this didn't happen.
Quanta of light
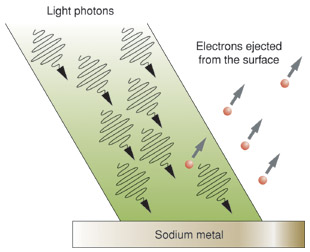 |
Photoelectric effect
|
Several years went by before Lenard's observations on the photoelectric
effect and Planck's strange but neglected theory of the quantum, both
puzzling in themselves, were seen as arrows pointing to a common
solution. Looking back now, it seems clear enough, but it took the
genius of Einstein to apply quantization, not to blackbody oscillators
as Planck had done in a desperate effort to patch up classical theory,
but to the actual radiation that's emitted or absorbed. Light itself
is quantized, Einstein realized. All the light of a particular
frequency comes in little bullets of the same energy, equal to the
frequency multiplied by
Planck's constant, and that's the key to understanding the
photoelectric effect. An incoming light quantum smashes into an electron
on the surface of a metal and gives up all of its energy to the
electron. A certain amount of energy, called the work function, is
needed simply to overcome the force of attraction between the electron
and the metallic lattice in order to set the electron free; so there
can't be any photoelectric effect unless this threshold is reached. Any
energy left over from the exchange, above and beyond the work function,
appears as kinetic energy (energy of motion) of the ejected electron.
Increasing the intensity of radiation – the number of light quanta per
unit area – has no effect on the energy of individual electrons because
each electron is thrown out by one and only one parcel of light.
Increasing the frequency of radiation, on the other hand, means that
each light bullet packs a bigger wallop, which results in a more
energetic photoelectron.
The fact that 16 years went by before Einstein won a Nobel Prize for his
groundbreaking work on the photoelectric effect, reflects how long it
took the scientific world to accept that radiant energy is quantized.
That may seem like an age, but the idea that energy, including light, is
granular ran counter to everything that physicists had been taught for
several generations: matter is made of particles; energy is continuous
and tradable in arbitrarily small amounts; light consists of waves;
matter and light don't intermingle. These rules had been the mantras of
physics for much of the 19th century and were now being overturned.
There was also the issue of experimental proof. It took a decade or so
for the details of Einstein's photoelectric theory to be thoroughly
tested and verified in the lab. The actual observation that the kinetic
energy of electrons kicked out by the photoelectric effect is tied to
the frequency of incoming light in exactly the way Einstein prescribed
was finally made in 1916 by the American physicist Robert Millikan.
Millikan had, in fact, long been expecting to prove Einstein wrong and
thereby to uphold the wave theory of light. Instead he wound up giving
powerful support to the particle theory and measuring Planck's constant
to within 5 percent of its currently accepted value. Ironically, he won
the Nobel Prize in 1923 for a superb series of experiments that dashed
what earlier had been his greatest scientific hope.
Slow revolution
We talk about the quantum revolution – but it wasn't an overnight
affair, this overthrow of the old worldview of matter and energy in
favor of new one. It was more than two decades after Planck's first
inkling of the existence of quanta when quantum theory was fully
accepted and acknowledged as the reigning paradigm of the microcosmos.
For the first part of this interregnum, Einstein was at the cutting edge
of developments. Following his seminal 1905 photoelectric paper, he
worked on meshing Planck's notion of the quantum with other areas of
physics. For instance, he showed that some anomalies to do with how much
heat substances have to absorb to raise their temperature by a certain
amount are best explained if the energy of vibration of atoms is assumed
to be quantized. This early quantum pioneering by Einstein now seems
almost entirely overshadowed by his work on relativity, but it was
instrumental at the time in persuading scientists of the validity of
quantum theory when applied to matter.
His views on the quantum nature of electromagnetic radiation proved a
harder sell. Yet, he insisted that the way ahead had to lie with some
acceptance of light's particlelike behavior. In 1909 he wrote: "It is my
opinion that the next phase in the development of theoretical physics
will bring us a theory of light that can be interpreted as a kind of
fusion of the wave and emission theory." In 1911, at the first Solvay
Congress (an annual meeting of the world's top physicists) he was more
forceful: "I insist on the provisional character of this concept, which
does not seem reconcilable with the experimentally verified consequences
of the wave theory." That apparent irreconcilability was a major
stumbling block for all scientists. What kind of madness was it to argue
that light could be both a particle and a wave?
Experimentalists railed at the prospect of what Einstein's equation of
the photoelectric effect implied. Robert Millikan, the very man who
showed that the equation really did work, would have nothing to do with
its physical interpretation. In 1915, Millikan wrote: "The
semicorpuscular theory by which Einstein arrived at his equation seems
at present wholly untenable." Three years later, Ernest
Rutherford, the great New Zealand physicist who probed the structure
of the atom, said there appeared to be "no physical connection" between
the energy and frequency in Einstein's hypothesis about light quanta. It
didn't seem to make sense that a particle could have a frequency, or
that a wave could act as if it were made of energetic particles. The two
concepts seemed to rule each other out.
Final proof of the particle nature of light
Between 1911 and 1916, Einstein took a sabbatical from his quantum work
to attend to another little problem – the general theory of relativity,
which transformed our ideas on gravity. Upon his return to the physics
of the very small, he quickly grasped a link between quantum theory and
relativity that convinced him of the reality of the particle aspect of
light. In earlier work, Einstein had treated each quantum of radiation
as if it had a momentum equal to the energy of the quantum divided by
the velocity of light. By making this assumption he was able to explain
how momentum is transferred from radiation to matter – in other words,
how atoms and molecules are buffeted when they absorb radiation.
Although this buffeting was much too small to be seen directly, it had
effects on properties, such as the pressure of a gas, that could
be measured. These measurements fitted with the formula for quantized
momentum. Einstein now realized, in coming back to his quantum studies,
that exactly the same expression for the momentum of a light quantum
fell straight out of a basic equation in relativity theory. This link
between relativity and the earlier assumption about the momentum of a
radiation quantum clinched the case for light particles in Einstein's
mind. In 1917, he may have been the only major scientist alive who
believed that light had a genuine particle aspect. But the fact that his
theory now insisted that whenever these supposed light quanta interacted
with particles of ordinary matter a definite, predictable amount of
momentum should be transferred, paved the way for experimental tests.
Six years later, the particle nature of light had been put virtually
beyond dispute.
At the heart of lab work that ultimately proved the reality of radiation
quanta was the American physicist Arthur Compton. In his early days at
Princeton, Compton devised an elegant way of demonstrating Earth's
rotation, but he soon launched into a series of studies involving X-rays
that climaxed in the final victory of quantum physics over the old world
order. In his mid-twenties Compton hatched a theory of the intensity of
X-ray reflection from crystals that gave a powerful tool for studying
the crystallographic arrangement of electrons and atoms in a substance.
In 1918 he began a study of X-ray scattering that led inevitably to the
question of what happens when X-rays interact with electrons. The key
breakthrough came in 1922 and was published the following year. Compton
found that when X-rays scatter from free electrons (electrons not
tightly bound inside atoms) the wavelength of the X-rays increases. He
explained this effect, now known as the Compton effect, in terms of
radiation quanta colliding with electrons, one quantum per electron, and
giving up some of their energy (or momenta) in the process. Energy lost
translated to frequency decrease, or wavelength increase, according to
the Planck formula. A further boost for this interpretation came from a
device invented by Charles Wilson. Inspired by the wonderful cloud
effects he'd seen from the peak of Ben Nevis, in his native Scotland,
Wilson built a vessel in which he could create miniature artificial
clouds. This cloud chamber proved invaluable for studying the behavior
of charged particles, since water droplets condensing in the wake of a
moving ion or electron left a visible trail. Wilson's cloud chamber
revealed the paths of the recoil electrons in the Compton effect,
showing clearly that the electrons moved as if struck by other particles
– X-ray quanta – which, being uncharged, left no tracks. Final proof
that the Compton effect really was due to individual X-ray quanta
scattering off electrons came in 1927 from experiments based on the
so-called coincidence method, developed by Walter Bothe. These
experiments showed that individual scattered X-ray quanta and recoil
electrons appear at the same instant, laying to rest some arguments that
had been voiced to try and reconcile quantum views with the continuous
waves of electromagnetic theory. To complete the triumph of the particle
picture of light, the American physical chemist Gilbert Lewis coined the
name "photon" in 1926, and the fifth Solvay Congress convened the
following year under the title "Electrons and Photons."
Doubt had evaporated: Light could manifest itself as particles. But
there was equally no doubt that, at other times, it could appear as
waves. And that didn't seem to make any sense at all. As Einstein said
in 1924, "There are ... now two theories of light, both indispensable
... without any logical connection."
|
|